CRISPR Part I: The DNA Swiss Army Knife
What would you do with the power to edit the code of life?
Welcome to Space Race, where we explore the impact of breakthroughs in science research on the intensifying global contest of superpowers in the 21st century. Subscribe for weekly breakdowns of the scientific breakthroughs that will shape the future.
Today’s newsletter is part 1 of 2 on CRISPR, an amazing technology that can edit genes with unmatched precision and speed. We’ll break down the basics of the biology to explain how CRISPR actually works and how it was cleverly repurposed from its original role as an immune system in bacteria. As always, we’ll also explore broader impacts, including how CRISPR could address food insecurity and wipe out entire species. Next week, we will focus on the possibilities for CRISPR in humans as well as the high stakes ethical questions raised by human applications.
There are a few reasons scientists do what we do. Many scientists would say they are driven by a relentless internal curiosity about nature and its secrets. Others would say that while curiosity is important, it’s the ability to harness nature’s secrets for human benefit that drives them. Today, nowhere is this second motivation more visible than in the field of biotechnology, which is undergoing a full renaissance that could impact almost every aspect of our lives. The pièce de résistance of the biotech renaissance is CRISPR — a gene editing technology that could grant us the unthinkable powers of eradicating disease and controlling human evolution.
A (Very) Brief Introduction To DNA & RNA
This section is included for those who’d like a primer of how DNA/RNA works, but can be skipped if you are comfortable with your ATCGs.
Before tackling CRISPR, let’s remind ourselves of the basics of genetics. The focal point of genetics is an organic molecule called deoxyribonucleic acid. You probably know it as DNA. This molecule carries the instructions for the growth, function, and reproduction for all life on Earth.
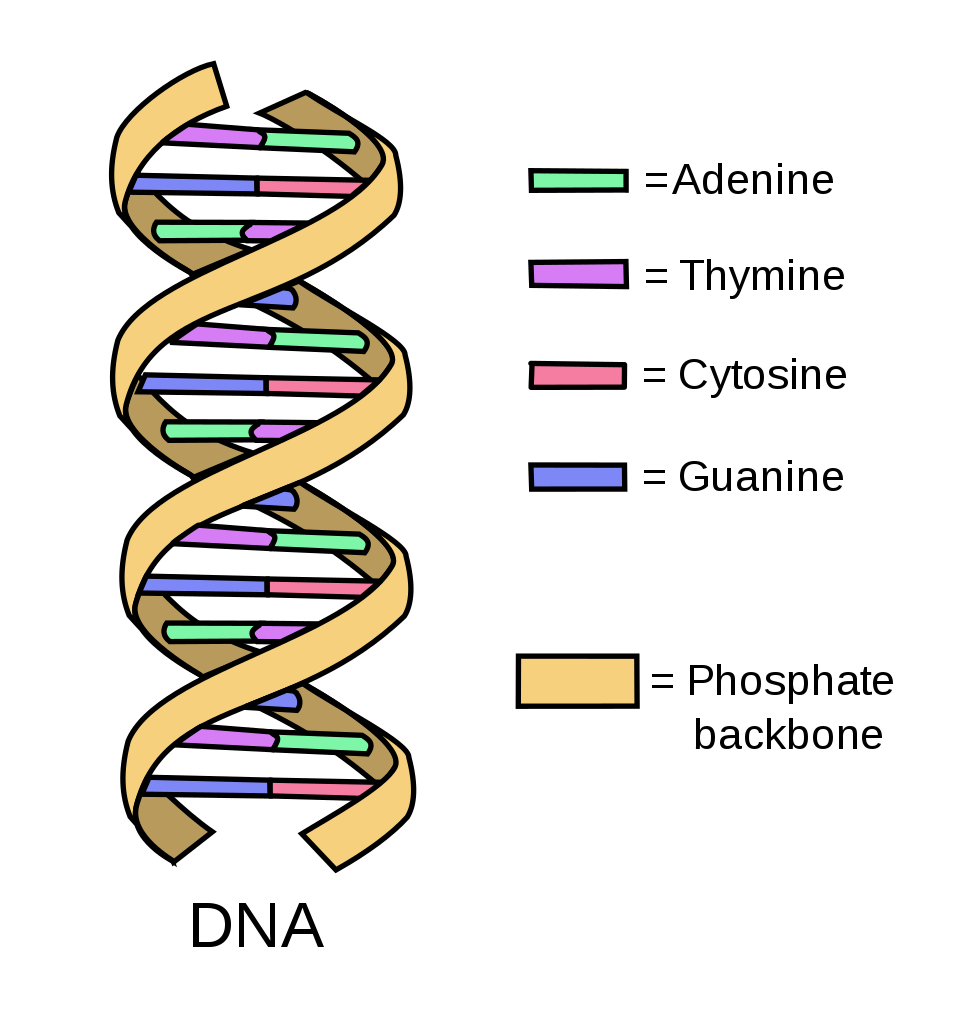
DNA is famous for its signature double helix structure. Each of the two phosphate strands of this double helix hold molecules known as nucleotides or bases. There are 4 nucleotides in DNA:
Adenine (A)
Thymine (T)
Cytosine (C)
Guanine (G)
The order in which these bases occur on the phosphate strands is how DNA codes for biological functions. Consider a very simplified example where the sequence AGTGGAC in some gene might code for blue eyes, while AGTGGAA might instead code for brown eyes. The key point is that this genetic code, which arises from the ordering of bases in a DNA molecule, is the fundamental set of instructions for all biological function.
Both strands of the double helix hold their own sequences of nucleotides, but these are not random pairs. In fact, both strands contain exactly the same information. This is because the nucleotides will only pair according to a very simple set of rules: A joins with T and C with G. This means that if one knows all the bases on one side of the double helix, they necessarily know the bases on the other side too. If one side contains the sequence AGTGGAC, we know the other side must contain TCACCTG. The reason for this redundancy is to provide the cell with a template for DNA replication and repair.
A very important supporting character in this story is DNA’s cousin RNA. While DNA is the genetic blueprint for all biological function, RNA is the means by which that blueprint is translated into actual biological proteins. RNA is created from a particular segment of DNA in a process known as transcription. In this, the DNA is unzipped and RNA is created by matching bases to one side of the double helix. One small difference is that where an RNA should have T — opposite an A on the DNA double helix — it will instead have a base called uracil (U).1
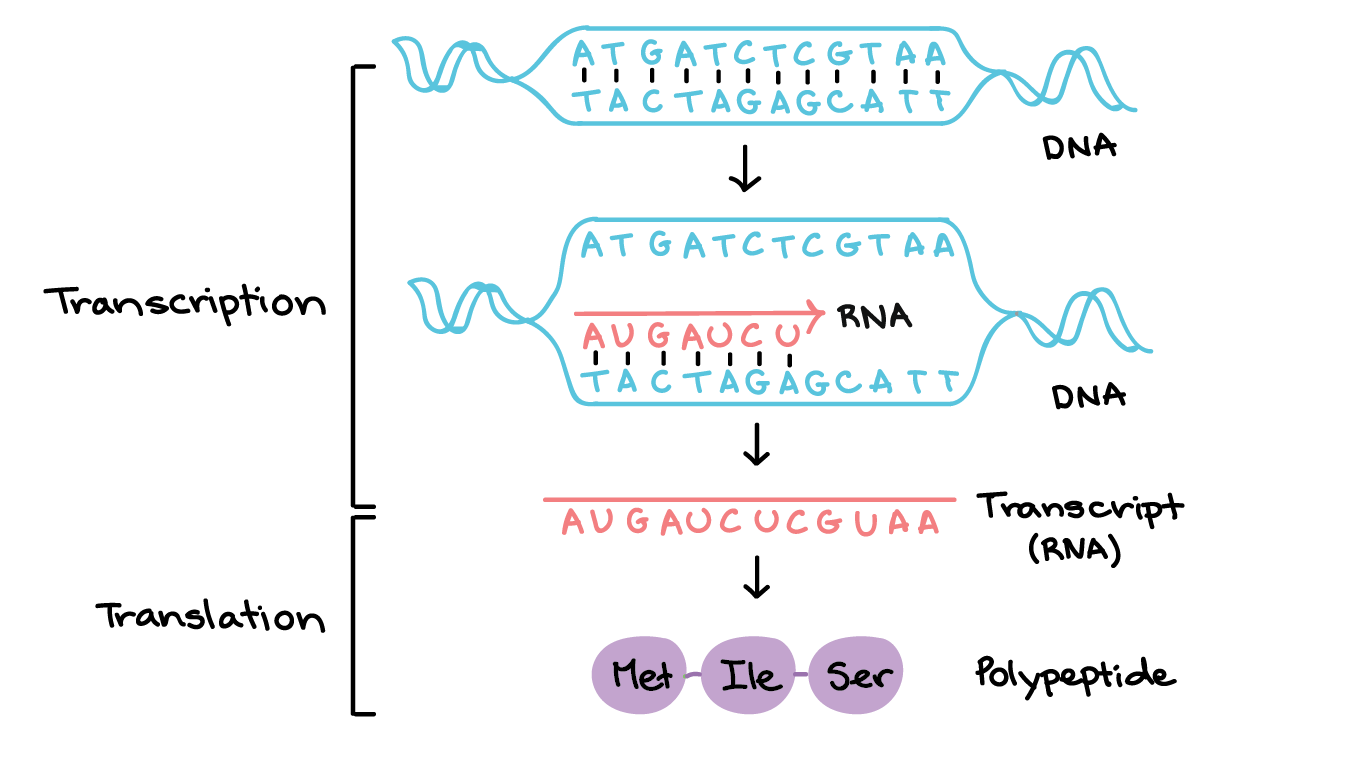
Another difference is that RNA is always single stranded since it isn’t as important as DNA. If RNA is damaged it can always just be recreated as long as there is viable DNA still available.
Once the RNA strand is made, it is used by the cell to create the proteins coded for in the DNA. This step of the process is known as translation. These proteins then give rise to biological function.
Enter CRISPR (And Cas9)
Once we discovered DNA and understood that it was the blueprint of biological life, a natural question to ask was how do we tinker with that blueprint. This gave rise to the field of genetic engineering. For decades, geneticists devised various methods to edit genes, each more accurate than the other. Two prominent examples are TALENs and Zinc Finger Nucleases. These were groundbreaking in their own right, but weren’t as versatile as scientists would have liked. For instance, TALENs can only induce one genetic change at a time, and both of them can be expensive and difficult to use.
CRISPR is the newest and most versatile addition to the gene engineering toolkit, discovered by Jennifer Doudna and Emanuelle Charpentier in 2012 and recognised by the Nobel Prize in Chemistry in 2020. The community had actually known about CRISPR in some form for far longer but it was Doudna and Charpentier who fully realised its capacity for gene editing.
CRISPR was originally discovered when scientists were analysing bacteria. They observed that many bacterial DNA sequences had a peculiar structure marked by repeating segments about 20-40 base pairs long. The bases in these sequences were also palindromes, meaning that they read the same backwards and forwards e.g. ATCGCTA. These repeating palindromic sequences occurred with a strange regularity, leading to their name: Clustered Regularly Interspaced Short Palindromic Repeats — hence CRISPR.

Scientists also observed two other important facts. First, the DNA between these repeating sequences matched the DNA of the viruses that attack bacteria. Secondly, the bacteria that did have the CRISPR sequences had higher immunity to viral attack. Putting two and two together, scientists concluded that this was a bacterial immune mechanism. But how did this viral DNA make it into the bacterial genome in the first place, and how did the bacteria use it to fight off infections?
The answer lies with a series of special proteins that help CRISPR, named CRISPR associated (Cas) proteins. These proteins exist in various forms and have varying functions that aid the bacterial immune response.
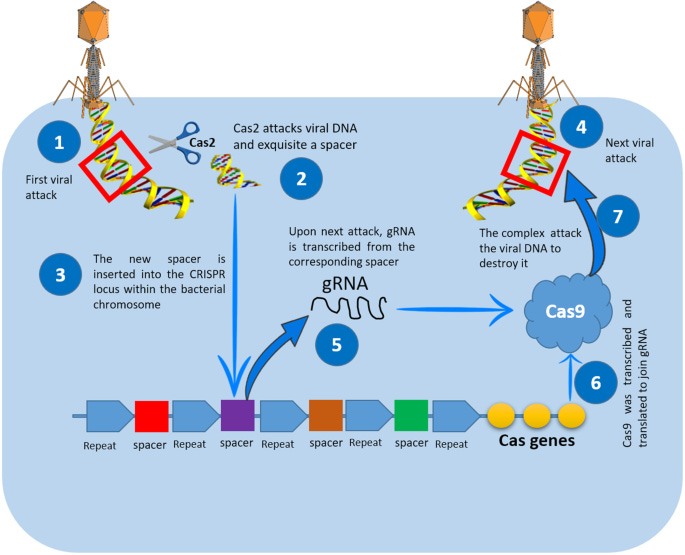
The basic CRISPR-Cas immune response is as follows:
A virus attacks and releases its DNA into the bacteria.
A Cas protein (called Cas2) will try to cut the foreign DNA and make a spacer sequence out of the cut segment.
This sequence is inserted into the bacterial DNA between two CRISPR repeats.
The same virus attacks again, activating the CRISPR defense mechanism.
The appropriate CRISPR spacer sequence is read to make a special RNA that identifies the viral DNA. This is called guide-RNA or gRNA.
The gRNA attaches to another Cas protein — Cas9 — and guides it to the viral DNA.
Cas9 cuts the viral DNA at the specific point targeted by the gRNA. The viral threat is removed.
This is no doubt a sophisticated and beautiful biological mechanism, but what has it got to do with gene editing?
The key lies in the precision with which the Cas9-gRNA complex cuts the viral DNA. By tremendous good fortune, nature just happened to give us a mechanism that can cut DNA with pinpoint accuracy given the appropriate gRNA. It isn’t perfect, but it’s still phenomenally accurate and unlike Zinc Finger Nucleases, it’s simple enough for anyone to toy with at home.
The central leap that turns Cas9 from a defense mechanism to a gene editing tool is to realise that we can simply give it whatever gRNA we want. Once we know what sequence of DNA we want to cut, we make a matching piece of gRNA in the lab and let Cas9 work its magic.
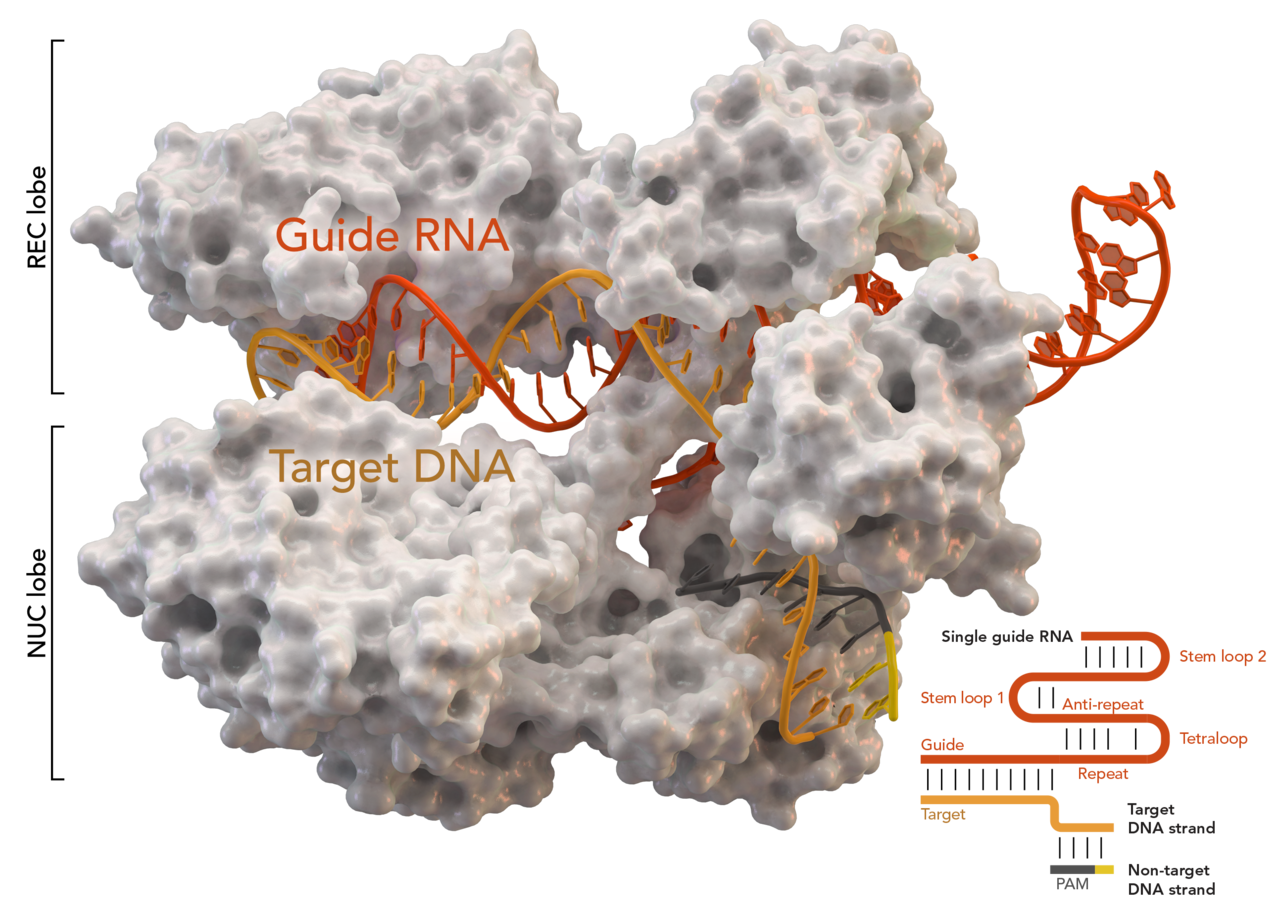
Gene Editing with CRISPR-Cas9
The simplest type of gene editing possible with CRISPR-Cas9 is to simply switch off unwanted genes. Once Cas9 cuts the targeted DNA sequence, the cell will try to repair it, but this process (called Non Homologous End Joining — NHEJ) is error prone and typically results in that gene just becoming deactivated.
If we want to instead replace the targeted DNA sequence with another sequence, we can give Cas9 our replacement sequence in addition to its gRNA. Once Cas9 makes the targeted cut, the cell will attempt to repair the broken DNA but this time it can reference the template DNA we have provided to make a more sophisticated repair than NHEJ. This process is known as homology directed repair (HDR).
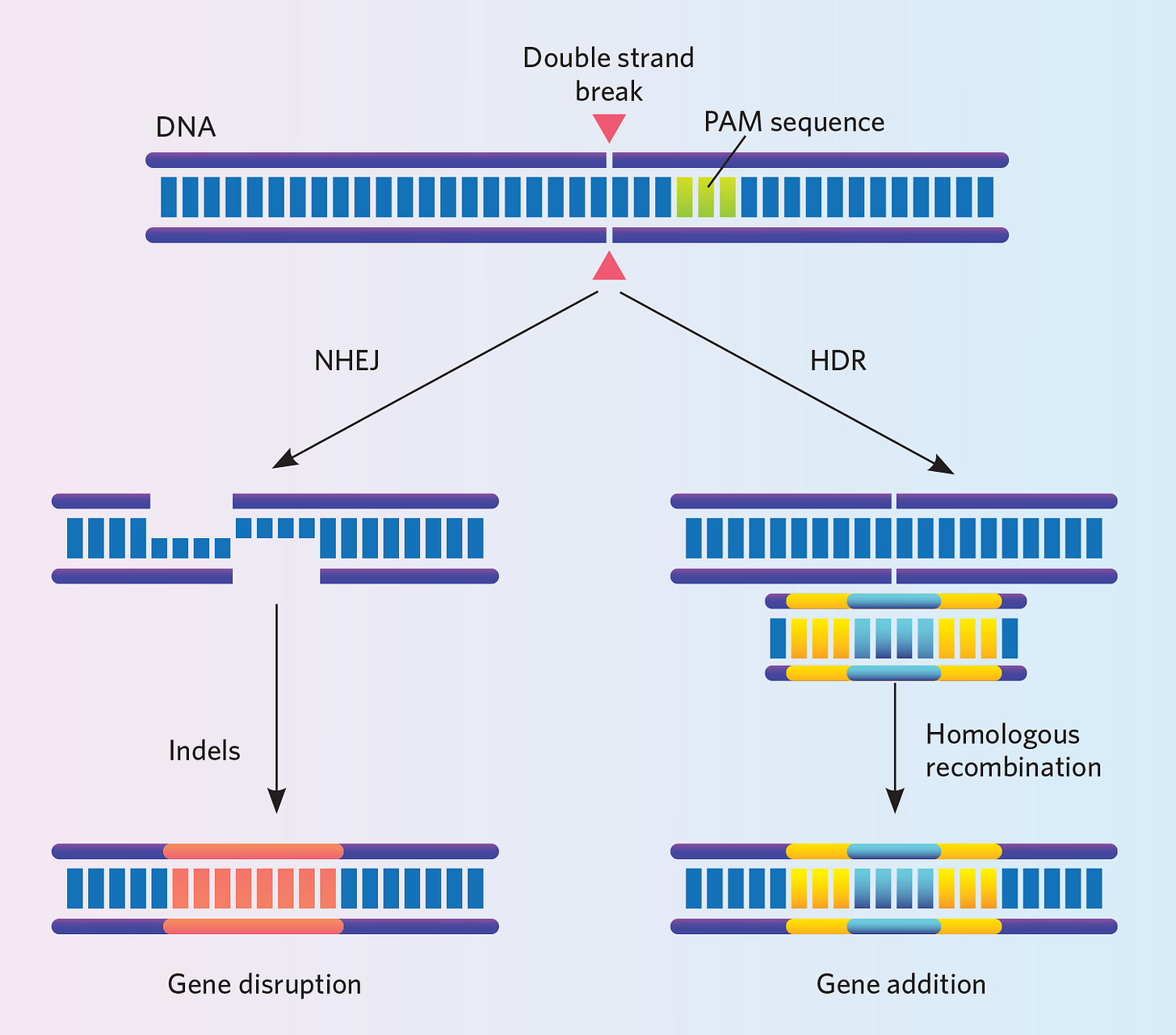
These are the most basic types of possibilities with CRISPR and since it is such a recent discovery, scientists are constantly developing new and exciting ways to use it. By allowing scientists to edit the blueprint of life as easily as a writer edits a book, CRISPR-Cas9 has led to an explosion in not only scientific research but has become a crown jewel of the current biotech renaissance.
CRISPR Beyond the Lab
The biotech renaissance is hardly confined to genomics laboratories, and countries around the world are beginning to realise the immense potential of CRISPR.
One important application of CRISPR is in agriculture, which has been taking advantage of genetic engineering ever since its inception almost 10,000 years ago. For millennia, farmers have been choosing the most desirable crops and livestock and selectively breeding them to make their genes more dominant in the population. Despite this, selective breeding was still more or less a game of chance since there was no way to ensure which traits would be passed on. The only guarantee was that over multiple generations, the desired traits would eventually become dominant.
With CRISPR, we can accelerate this process to produce drastic change as early as the current generation. Consider wheat, which is one of the most important staple foods of the world. A huge problem that farmers face is powdery mildew, a fungal disease that affects wheat and many other plants. The vulnerability to powdery mildew in these comes from the MLO gene. With selective breeding, farmers would have first needed to be lucky to find a few wheat plants without this gene, breed them selectively and hope that their resistance becomes widespread over generations. With CRISPR, it’s simply a matter of cutting MLO with Cas9 and deactivating it. CRISPR has also been used make rice grow more easily in cold weather, which is hugely significant as rice is the most widely consumed staple food in the world. Severe food insecurity already affects almost one billion people over the world and climate change threatens to make it worse. Given this, it’s hard to see how CRISPR-modified crops won’t become an increasingly indispensable part of diets worldwide.
Controlling Evolution with Gene Drives
One of the most powerful and terrifying capabilities of CRISPR is to fundamentally alter the rules of heredity itself and change the way in which genes are passed down. This is done via a technique known as a gene drive.
Animals usually have two copies of most of their genes — one from each parent. During reproduction, only one copy of the gene is passed on. This means that any gene has a 50% chance of being inherited. If we edit one of the genes, our CRISPR mutation only has a 50% chance of being passed on. With these odds, the mutation might disappear altogether from the population unless we can edit a large number of individuals at the same time. This could work for livestock in a herd, but for something like malaria carrying mosquitoes this is impossible.
But what if we use CRISPR to insert not only the mutation we want, but also implant CRISPR DNA itself into the target genome? More precisely, suppose that this CRISPR sequence targets the normal copy of the gene that wasn’t edited before. When the mutated gene drive is read by the cell, the CRISPR DNA will direct Cas9 to cut the other normal copy of the gene and disable it. When the cell attempts to repair this, the CRISPR mutated gene is available as a template, so the cell repairs the damage by replicating it. Voilà! Now we have two copies of the mutated gene, and inheritance is guaranteed.
The awesome power of gene drives lies in the fact that as long as one member if a population has the gene drive mutation, all members will inevitably get it. The CRISPR mutation will (almost) always replicate itself and guarantee inheritance.
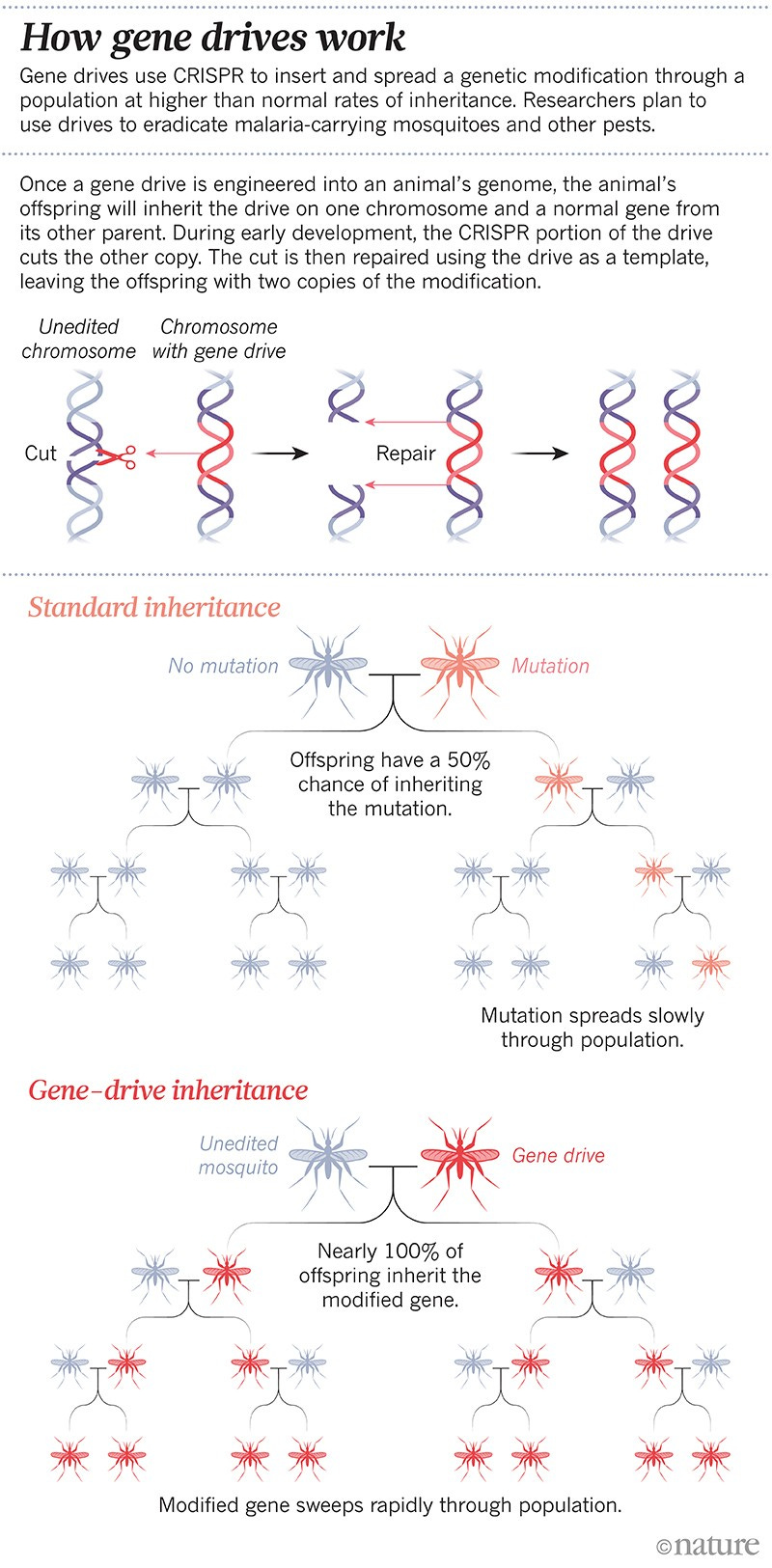
So far, gene drives have not been used beyond the lab, but many are already contemplating unleashing them on malaria spreading mosquitoes in Africa. With the right mutation, a gene drive could render these mosquitoes harmless, or eradicate their species entirely.
There is something terrifying about having such total control over evolution. While gene drives could clearly cause great benefit, there is huge potential for misuse. Even if used in good faith, gene drives could have disastrous unintended consequences on entire ecosystems. At this early stage, it is difficult to imagine how nature would respond to such a flagrant intrusion by humans into its domain of control.
Conclusion
Discovered barely a decade ago, CRISPR-Cas9 is arguably one of the most important discoveries of the 21st century. Originally crafted by nature to act as an immune system in bacteria, CRISPR has been repurposed to edit genes in any organism with unmatched precision and speed. With the ability to edit the blueprint of life as a writer would a text document, humans can now seriously claim to be able to direct evolution according to their desires. Such unthinkable power has already had very real impacts. It could be the decisive weapon in the fight against food insecurity and diseases such as malaria. It could also be used to accidentally destroy entire ecosystems and irreversibly damage the delicate equilibrium cultivated by natural selection over millions of years if we misuse its power. There are almost certainly many more applications for CRISPR to come, each carrying a comparable risk-reward tradeoff with it. Given this, it is imperative that we pause and ensure that we fully understand the tremendous responsibility that comes with the power to rewrite the code of life.
Especially if we plan to use it on ourselves.
Next week, we will explore the consequences of using CRISPR in humans, such as curing genetic diseases like cystic fibrosis and making designer babies. We will also consider the deep ethical issues associated with rewriting human DNA, especially DNA that can be inherited, and how attitudes to CRISPR research differ around the world as countries attempt to balance ethics with innovation.
Thymine and uracil are very similar molecules with minor differences. The main reason for using uracil in RNA is that it is easier to produce and since RNA is made more often DNA, it is energetically favourable to just use uracil. Why not use uracil in DNA also? Because cytosine (C) sometimes converts into uracil. So if uracil were a DNA base, and one of the C bases degraded into a U, the cell won’t be able to tell if that U was supposed to be there anyway or if it was a degraded C. By using T, there is no confusion — if a C degrades into a U it must be fixed. If a C becomes a U in RNA, it’s not so important. The RNA will be unviable but we can quickly make a new copy as long as we have access to the DNA. It is the greater importance of DNA over RNA that incentivises nature to use a T in DNA but a U in RNA.